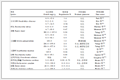

Review on the abiotic degradation of biodegradable plastic poly(butylene adipate-terephthalate): Mechanisms and main factors of the degradation
English
Review on the abiotic degradation of biodegradable plastic poly(butylene adipate-terephthalate): Mechanisms and main factors of the degradation
-
Key words:
- Biodegradable plastics
- / PBAT
- / Abiotic degradation
- / Degradation mechanism
-
1. Introduction
In recent years, the global plastic production has witnessed a notable increase as reported by Plastics Europe, and it is anticipated to continue growing. This surge in production has led to a substantial influx of plastic waste into the environment, where it accumulates. The difficulty in degrading most plastics due to being petroleum-based materials has resulted in an adverse impact on the global environment, driving extensive research into the degradation of plastics [1,2]. Consequently, there has been a significant focus on biodegradable plastics (BPs) due to their enhanced degradability, bioaffinity, and oxidation properties. These BPs differ significantly from ordinary plastics as they can be broken down into small molecules (such as water, carbon dioxide, methane, and biomass) by microorganisms under specific conditions [3-5]. Among the biodegradable plastics, poly(butylene adipate-terephthalate) (PBAT) has garnered particular attention due to its complete biodegradability and high flexibility, with an elongation fracture rate of up to 710% [6,7]. It is widely utilized in various fields, such as packaging, agriculture, and biomedicine. Furthermore, it is worth noting that PBAT's production has been the primary driver behind the growth of biodegradable plastics in the past five years [8].
PBAT is a copolyester composed of aliphatic and aromatic groups, and the properties of this material are closely related to the ratio of these two raw materials. As the aliphatic chain segments increase, the material's elongation at fracture first increases and then decreases, while the tensile strength exhibits the opposite trend. Moreover, higher aliphatic chain segments also lead to increased sensitivity to erosion [9]. Additionally, in the production process, parameters like mold temperature, injection speed, and pressure will impact the mechanical properties of PBAT. Experimental evidence demonstrates that PBAT composites subjected to lower mold temperatures and injection speeds exhibit increased flexural strength and tensile strength [10]. In addition to controlling the raw material ratios and process parameters, adding additives to PBAT is an effective means of modification. For example, the addition of oxalic acid can alter the aliphatic unit of PBAT, providing stronger gas barrier, hydrophilicity, and degradation ability [11]. Furthermore, to enhance mechanical properties, reduce costs, and accelerate degradation, PBAT blends with poly(lactic acid) (PLA), starch, and various nanoparticles are utilized [12-15].
PBAT waste undergoes natural weathering, mechanical wear, hydrolysis, photochemical transformation, and microbial degradation. However, the limited variety, quantity, and activity of microorganisms in the natural environment means that aged PBAT cannot be fully degraded [16-18]. Therefore, to achieve complete degradation of most waste plastics, industrial methods such as landfilling, composting, pyrolysis, or incineration are employed [19]. The degradation of PBAT waste can be divided into two categories: abiotic degradation (physical and chemical degradation) and biotic degradation. The former uses microorganisms or other organisms to degrade PBAT into carbon dioxide and water, while the latter degrades PBAT into small molecules by various abiotic means. In terms of degradation rate, biodegradation is slow and abiotic degradation is fast. However, considering energy consumption, biological degradation is superior to abiotic degradation techniques [20]. It is worth noting that abiotic degradation often precedes biodegradation, for example, PBAT waste needs to be converted into small molecules first and then absorbed by microorganisms [16]. However, the so-called “precedes” here is not a strict sequence relationship, as PBAT waste can undergo multiple degradation reactions simultaneously in both natural and industrial environments. For instance, in ocean, hydrolysis, photo-oxidative degradation, and biodegradation often occur concurrently [16,21]. Therefore, combining both degradation methods shows great promise. However, few reports have systematically investigated the abiotic degradation of PBAT [6,22-24]. Water, light, and heat are widely present natural elements in the environment. Studying their impact on the degradation of PBAT is of significant practical importance, as the degradation caused by them occurs everywhere in the natural environment. Although PBAT also undergoes abiotic degradation through other pathways, these alternative abiotic degradation pathways are undoubtedly inferior in practical applications. Furthermore, compared to the biodegradation pathway, existing research lacks in-depth exploration of abiotic degradation mechanisms and discussion of the factors influencing abiotic degradation.
Based on the above discussion, this work aims to provide a comprehensive review of current research on the abiotic degradation of PBAT, with a focus on summarizing the hydrolysis, photochemical transformation, and thermochemical degradation processes. Additionally, it will briefly introduce the basic synthesis methods and properties of PBAT, and analyze the influence of raw material ratio and process parameters on its degradation. The main objective of this study is to establish a theoretical foundation and offer practical guidance for identifying an economical, efficient, and environmentally friendly approach for managing PBAT waste.
2. Characteristics and variations of PBAT
PBAT, a copolymer, is composed of two chemical units: butanediol adipate (BA) and butanediol terephthalate (BT). Its synthesis entails the condensation of three raw materials, namely 1,4-butanediol, adipic acid, and terephthalic acid [25], with the ratios of these raw materials impacting the processing conditions, commercial application, and end treatment of PBAT. Various studies have delved into the effects of different BA to BT ratios on PBAT's characteristics and degradability. For instance, Herrera et al. compared three PBATs with different BA to BT ratios (2:3, 1:1, and 3:2) and found their thermal properties and degradability to be closely linked to these ratios [26]. It was observed that the melting point, crystallinity, glass transition temperature, and tensile modulus increased with higher BT content, while the hydrolytic degradation rate and enzymatic degradation rate depended largely on BA content. Furthermore, research by Wang et al. revealed that an increase in BT content led to a less favorable enzymatic degradation of PBAT [27]. The Kijchavengkul et al. research also concluded that compared to the rigid aromatic domain (BT) and the crystalline region, the soft aliphatic domain (BA) and the amorphous region are more vulnerable to hydrolysis and biodegradation [28].
The changes in surface morphology and chemical structure of PBAT after degradation are worthy of attention. Using scanning electron microscope (SEM) and differential scanning calorimetry (DSC) can accurately characterize the changes in PBAT before and after degradation [26,29-35]. If ultraviolet (UV) radiation or freshwater with sediment are involved in PBAT degradation, the surface of the PBAT sample becomes rough, generating numerous corrosive holes, and the position of the infrared absorption peak also changes [33,34]. The increase in the melting point of PBAT with the progression of degradation is attributed to the degradation of aliphatic BA, leading to a higher proportion of the crystalline structure of BT [33]. Furthermore, the O/C content ratio in PBAT increased during natural hydrolysis, rising from 0.37 at the beginning to 1.44 after 24 months. This ratio increased rapidly in the first 12 months, followed by a slower increase in the subsequent 12 months (Table 1). This observation can be attributed to the ester bonds' degradation by hydrolysis, resulting in a higher number of carboxyl groups in the molecule, consequently increasing the O element content. Moreover, hydrolysis is demonstrated to occur preferentially in the non-crystalline region and proceeds more rapidly in the first 12 months, leading to a more pronounced change in the O/C content ratio [28].
Table 1
Table 1. Proportions of different components of PBAT before and after degradation [33].In addition to the changes in surface morphology and chemical structure, the toxicity of PBAT also warrants attention. Recent researchers have discovered that due to the biodegradability of PBAT, bacterial communities are more easily attached to its surface, leading to a potential elevated risk of the spread of antibiotic-resistant genes [36]. Hence, while the oligoesters or monomers resulting from PBAT degradation do not display clear toxic effects, further research is necessary to assess whether the benefits of using PBAT in substantial quantities justify the drawbacks.
As shown in Fig. 1 [23,37,38], we have conducted a brief summary on factors that can influence the degradation of PBAT. Based on the relationship between factors and PBAT, the factors affecting PBAT degradation can be divided into two categories: endogenous and exogenous factors. Endogenous factors are closely related to PBAT, mainly referring to the properties of PBAT itself, such as molecular weight, hydrolysis rate, crystallinity, and hydrophilicity. Exogenous factors mostly originate from the external environment, including product concentration, temperature, ultraviolet light, microbial species, and content. Similarly, we can classify the influencing factors as biotic or abiotic, depending on whether they are biological or not. The ratio of synthesized monomers being discussing in this section belongs to exogenous factors, and the specific effects of more endogenous and exogenous factors are discussed in the following section.
Figure 1
3. Abiotic degradation of PBAT
The degradation of PBAT in the environment encompasses both abiotic and biotic pathways. This dichotomy hinges on the involvement of organisms. Biotic degradation involves the breakdown of plastic polymers into H2O, CO2, CH4, and biomass due to the activity of microorganisms, differing from abiotic degradation [39]. Both abiotic and biotic degradation involve physical and chemical processes. Physical changes primarily manifest at the material level, such as rupture and embrittlement, while chemical changes occur at the molecular level, including breakage and the formation of chemical bonds [40]. Four commonly utilized methods to assess PBAT degradation are macroscopic observation, mass loss measurement, evaluation of changes in rheological properties, and detection of degradation products [41,42]. Each method differs in its approach to evaluating degradation and should be applied based on research objectives. This section will concentrate on the degradation mechanisms, influencing factors, and current research on hydrolysis, photochemical transformation, and thermochemical degradation, aiming to address the gap in the systematic summary of PBAT's abiotic degradation.
3.1 Hydrolysis
Hydrolysis, a crucial mechanism for the degradation of biodegradable polymers (BPs) due to the presence of hydrolyzable covalent bonds, such as ester bonds, ether bonds, and anhydrides, is a significant process influenced by the widespread occurrence of water in the environment [40]. As an aliphatic/aromatic polyester compound, PBAT is bound to be hydrolyzed by the presence of ester bonds (-COO). In the presence of water, these ester bonds are susceptible to attack and subsequent cleavage by water molecules [43]. This hydrolytic cleavage results in the formation of new hydroxyl and carboxyl groups at each end of the PBAT molecular chain, leading to a reduction in chain length (Fig. 2).
Figure 2
The hydrolysis of PBAT commences from the amorphous region, and then the crystalline region will begin to degrade [6]. The aliphatic chain segment will be hydrolyzed faster because of the existence of an amorphous region. As illustrated in Fig. 3, the hydrolysis of PBAT encompasses two erosion mechanisms: surface erosion and bulk erosion [44]. While the erosion principles at the molecular level are comparable for both mechanisms, surface erosion initiates degradation from the material's surface, while bulk erosion entails simultaneous degradation within the material and at the surface. When the rate of water diffusion into the material is lower than the rate of hydrolysis, surface erosion dominates the degradation process, with bulk erosion prevailing in the opposite scenario [45].
Figure 3
Deshoulles et al. hydrolyzed PBAT at different temperatures (80, 90, 100 ℃) [46]. The experimental results show that the hydrolysis rate of PBAT accelerated with the increase in hydrolysis temperature, which may be due to the intensified reaction between water molecules and ester bonds at high temperatures. In addition, they found that the ester bond located between BA and BT moieties would be hydrolyzed preferentially, followed by the internal ester bond of BA. Research has shown that certain bacteria can accelerate the degradation of PBAT because these bacteria can secrete lipases that promote the hydrolysis of PBAT [25,47,48]. For example, Jia et al. isolated Stenotrophomonas sp. YCJ1, a bacteria found in farmland, that secretes a lipase capable of recognizing and catalyzing the hydrolysis of the ester bond between BA and BT in PBAT, resulting in the formation of oligomers or monomers after several hydrolytic reactions [47]. The catalytic hydrolysis of other bacteria generally follows a similar scheme. Tang et al. found that the addition of a chain extender (e.g., Joncryl ADR 4370) can accelerate the degradation rate of PBAT [49,50]. When the quantity of chain extender is decreased, the molecular chain of PBAT is horizontally extended. This is because the epoxy group on the extender reacts with the terminal carboxyl group or hydroxyl group of PBAT to produce a hydrophilic hydroxyl group. The hydrophilic hydroxyl group accelerates the attack of water on the ester bond. On the other hand, with an increased concentration of chain extender, the PBAT chain becomes vertically interconnected, forming a network structure in addition to its horizontal extension (Fig. 4) [50]. In addition to the above factors, compounding PBAT with other materials can also affect its hydrolysis. For example, when it is compounded with PLA, the degradation rate of the composite is reduced compared to that of pure PLA or PBAT [33].
Figure 4
Figure 4. Reaction mechanism between PBAT with (a) low and (b) high content of ADR (a chain extender). Reprinted with permission [50]. Copyright 2021, Elsevier.Hydrolysis of PBAT can occur through surface erosion and bulk erosion, leading to the breakdown of the ester bond and carbonyl group [44,45]. Various factors such as higher temperatures, specific lipases, and certain additives can influence the hydrolysis rate of PBAT. It is noteworthy that these mechanisms have different effects. For instance, elevated temperatures can accelerate hydrolysis by providing additional energy, lipases can reduce the reaction activation energy through catalysis, and the addition of an extender can facilitate hydrolysis by altering the structure of PBAT [46,47,50].
3.2 Photochemical transformation
The carbonyl group and the benzene ring are two types of photosensitive groups in PBAT that easily initiate photocatalysis [49]. The carbonyl group is particularly sensitive to photochemical transformation and undergoes Norrish I and Norrish II reactions upon absorption of ultraviolet radiation energy [24,51] (Fig. 5a). In a Norrish I reaction, the adjacent carbon-carbon single bond (C-C) and carbon-oxygen single bond (C-O) to the carbonyl break, yielding benzene radicals and acyl radicals, which eventually form alkane radicals and acyl groups. Conversely, the Norrish II reaction involves the carbonyl group capturing a hydrogen atom at the γ-site, followed by the α-β C-O bond breaking to produce olefins and carboxyl groups. Both reactions lead to the main chain breakage of PBAT and its subsequent photochemical transformation. Subsequent to the Norrish I reaction, the intermediate products (benzene radical and acyl radical) enter into another crosslinking mechanism (Fig. 5b) [24,52,53]. The terephthalic acid ester reacts with these radicals, resulting in the destruction of the original structure and the formation of new products, namely benzophenone and biphenyl substances. Maurer-Jones et al. conducted fluorescence emission spectroscopy to measure the degradation of PBAT under three conditions (without UV, UV with oxygen, and UV without oxygen) [24]. The results demonstrate that the photochemical transformation of PBAT in the presence of oxygen is more complete, as photooxidation is another crucial mechanism contributing to the photochemical transformation of PBAT (Fig. 5c) [24,53,54]. In an oxygen atmosphere, the O-O bond of hydroperoxide breaks to form a hydroxyl radical, which subsequently replaces the hydrogen on the benzene ring in terephthalate to induce hydroxylation.
Figure 5
Figure 5. Mechanisms involved in the photochemical transformation of PBAT. Reprinted with permission [24]. Copyright 2021, American Chemical Society.Interestingly, different types of photochemical transformation of PBAT generate products with different molecular weights. According to Stloukal et al.'s photooxidation experiments on PBAT films, the rearrangement of PBAT chains by cross-linking would increase the molecular weight of PBAT, and this phenomenon becomes more obvious with the increase in the content of aromatic components [55]. Kijchavengkul et al. determined the molecular weight of PBAT film that had been dissolved in tetrahydrofuran (THF) after being photochemically converted [22]. Experiments have shown that the chain breakage and cross-linking of PBAT occur in the non-crystalline region under UV irradiation and that chain breakage reduces the molecular weight of PBAT (Table 2), while cross-linking will produce an insoluble polymeric gel [56].
Table 2
Table 2. Changes in molecular weight of the biodegradable films [22].In agricultural activities, PBAT film can preserve heat and moisture, inhibiting the breeding of pests and the reproduction of weeds, if its good mechanical properties preserved [57]. However, the growth of crops often requires a lot of sunlight, and PBAT film undergoes photochemical degradation under sunlight irradiation, resulting in the decline of mechanical properties, which is not conducive to the protection of crops. Therefore, it is desired to slow the photochemical degradation of PBAT films to achieve a longer service life. Several strategies have been proposed in research to slow down the photochemical transformation of PBAT. For instance, Yang et al. discovered that incorporating calcium carbonate (CaCO3) into PBAT under consistent weathering conditions effectively retards the degradation of PBAT. They found that the more uniformly CaCO3 is dispersed, the greater the effectiveness in slowing down photo-oxidative degradation. This was attributed to the ability of CaCO3 to block the penetration of ultraviolet rays, thereby reducing the photo-oxidative degradation of PBAT [58]. Additionally, Qiao et al. developed PBAT films with anti-UV aging by integrating new UV absorbers, allowing for controlled functional cycles by adding different UV absorbers [59]. Furthermore, the inclusion of carbon black has also been found to inhibit the photochemical transformation of PBAT [49].
The photochemical transformation of PBAT involves scission, crosslinking, and oxidation. Scission breaks the C-O and C-C bonds near the carbonyl group, yielding acyl groups and alkenes. Crosslinking increases the molecular weight of polymer segments, leading to the formation of an insoluble polymeric gel. Meanwhile, oxidation introduces hydroxyl groups into the original structure. These distinctions aid in identifying the specific type of photochemical transformation that has taken place in PBAT. Furthermore, measures to delay such transformations reveal that both CaCO3 and UV absorbers achieve their objectives by reducing the energy (light energy) capable of breaking down the polymer chain segments.
3.3 Thermochemical degradation
Thermochemical degradation of PBAT can be categorized into pyrolysis and thermal degradation. Pyrolysis is the decomposition of polymers at high temperatures under anoxic conditions to produce hydrocarbons of varying chain lengths for energy recovery [60]. Thermal degradation is a milder thermochemical reaction that can occur at lower temperatures and under aerobic conditions. The complex nature of PBAT pyrolysis has been summarized by Zhang et al., who identified four main mechanisms, consisting of end-chain cracking, side-chain cracking, random cracking, and chain cross-linking [20]. End-chain cracking and random cracking will decompose PBAT into corresponding monomers or fragments of uneven lengths, while chain cross-linking always occurs during the carbonization stage. The pyrolysis process can be categorized into slow pyrolysis, fast pyrolysis, and flash pyrolysis based on the speed of the heating rate, yielding four main products: liquid oil, pyrolytic gas, char, and wax [61]. In general, the composition of pyrolysis products is heavily influenced by the raw material; however, plastics typically yield predominantly liquid oil due to their high volatile content and low ash content. Furthermore, investigations into the pyrolysis of PBAT/PLA mulch film have indicated that the temperature and pyrolysis atmosphere play crucial roles in the process [62]. Specifically, when PBAT/PLA mulch film undergoes pyrolysis in a nitrogen or carbon dioxide environment, it has been observed that PBAT pyrolysis in the latter produces a greater quantity of monomer compounds and fewer polycyclic compounds, with higher pyrolysis temperatures resulting in increased yields of gaseous products. In terms of thermal stability, other researchers have shown that the addition of nanometals can improve the thermal stability of PBAT, due to a barrier effect of the nanoparticles toward polymer decomposition product ablation, which enhances the overall thermal stability of the system, making it less susceptible to pyrolysis, but this is limited to small additions (under 5% (w/w)). When the nanometal load was at 5% (w/w), agglomeration began to occur and the barrier effect decreased [31,63].
PBAT can be also degraded by thermal degradation, the difference between thermal degradation and pyrolysis is the operating temperature. The temperature required for thermal degradation is lower than that required for pyrolysis. For thermoplastics, this temperature is near the melting point, and for PBAT, the structure changes when the temperature exceeds the glass transition temperature [40]. There are two ways of thermal degradation to destroy PBAT: one is random chain breaking, and the other is terminal degradation [64]. The former reduces the molecular weight of the PBAT, and the latter leads to the production of volatile substances.
Al-Itry et al. (Fig. 6) concluded that the thermal degradation of PBAT occurs with β-C-H hydrogen transfer reactions in addition to the breakage of the main chain [42]. They also suggested that the thermal degradation of PBAT can be better assessed using functional group titration compared to Fourier Transform Infrared Spectroscopy (FTIR) spectroscopy. This is attributed to the increase in carboxyl group content of PBAT after degradation. Signori et al. investigated the effect of temperature on the thermal degradation of PBAT by heating it from 30 ℃ to 600 ℃. Their findings revealed that the molecular weight of PBAT remained unchanged when the temperature was less than 200 ℃, indicating that PBAT is thermally stable below 200 ℃ [65].
Figure 6
When oxygen is present, thermal degradation can be named thermo-oxidative degradation. The reactions involved in thermo-oxidative degradation and photo-oxidative degradations are similar, and both are classified as oxidative degradations. But the principal difference between the thermo- and photo-oxidative degradations is the sequence of initiation steps leading up to the auto-oxidation cycle; thermo-oxidative is thermally initiated and the other is photochemically initiated. In addition, selectivity is also different for the degradation pathways, thermal reactions occur throughout the bulk of the polymer sample, not just on the surface for the case of photo reaction [64,66]. Researchers have demonstrated that the rate of thermo-oxidative degradation is influenced by temperature when the oxygen concentration is maintained at 5%-20% [20]. Additionally, the use of catalysts has been explored to facilitate the thermal degradation of PBAT. For instance, Li et al. investigated the application of halloysite nanotubes as catalysts for the thermal degradation of PBAT, and their findings revealed that the addition of halloysite nanotubes altered the pyrolysis pathway of PBAT. Specifically, they observed that at temperatures below 200 ℃, PBAT underwent partial hydrolysis, followed by β-H transfer, and ultimately, the volatile gas was carbonized upon entering the lumen [67].
Although both pyrolysis and thermal degradation belong to thermochemical degradation and can lead to random breakage of the main chain of PBAT, the reaction temperatures and the influencing factors of pyrolysis and thermal degradation in PBAT are different. Pyrolysis is affected by temperature, pyrolysis atmosphere, and other factors, while thermal degradation is affected by temperature and catalyst. The temperature of thermal degradation is often lower than that of pyrolysis. In addition, the objectives of the two methods are different, pyrolysis is for energy recovery and material recovery, while thermal degradation aims to achieve an efficient treatment.
Through the observation of PBAT hydrolysis, photochemical transformation, and thermochemical degradation mechanisms, it becomes evident that each type of degradation leads to different “damages”. For instance, hydrolysis results in the destruction of the ester bond, photochemical transformation leads to the destruction of the carbonyl group, and thermochemical degradation can cause the destruction of C-C bonds. This indicates that identification of the degradation type is possible by detecting the changes in the functional group. The key to the abiotic degradation of PBAT is to use abiotic factors to break chemical bonds of PBAT, thus reducing the molecular weight of PBAT. By summarizing the mechanisms of influencing factors, it can be seen that different factors have a common “bond”. For example, temperature, light intensity, lipase, calcium carbonate, and nanometals are related to energy; changing the ratio of raw materials, and adding chain extenders to synthesize composite materials are related to the PBAT molecular structure. This can provide new ideas for the treatment of PBAT waste. Since multiple abiotic factors always coexist, these three degradations cannot happen independently. Therefore, it is more practical to study the combined effects of multiple degradation pathways, which are currently lacking. Notably, certain relationships exist between the main factors. For instance, photochemical transformation and thermochemical degradation, both associated with oxygen, and temperature, have an impact on all three degradations. Furthermore, certain substance additions can also affect these degradation processes. This suggests potential breakthroughs for future research.
4. Conclusion and prospect
This review offers an in-depth analysis of the abiotic degradation mechanisms of PBAT, focusing on hydrolysis, photochemical transformation, and thermochemical degradation, and their key influencing factors. The degradation of PBAT leads to significant changes in the molecular-level structure of the polymer chain segments. Moreover, the primary factors affecting these degradation processes, including temperature, additives, and catalysts, exhibit a commonality in their direct or indirect impact on the energy required for degradation or the structural integrity of PBAT. On this basis, we further draw two conclusions: (1) The common ground of hydrolysis, photochemical transformation, and thermochemical degradation is to preferentially destroy the chemical bonds with higher energy (especially C-O and C=O) of PBAT, which eventually leads to the shortening of the polymer chain and then leads to a reduction in molecular weight. (2) It is possible to alter the structure of PBAT or impacting the energy required for degradation reactions to affect the pace at which it degrades. Comprehending the mechanisms and primary factors of abiotic degradation of PBAT can furnish a theoretical framework and practical direction for identifying effective approaches to degrade PBAT waste.
Further research is essential to enhance our understanding of the abiotic degradation of PBAT, particularly focusing on mechanical-chemical degradation and reactive oxygen degradation. Additionally, it is crucial to conduct further studies to investigate the interactions between hydrolysis, photochemical transformation, and thermos-chemical degradation, as these factors often coexist during the degradation of PBAT. In conclusion, to broaden the application of PBAT materials and minimize environmental impact, it is imperative to intensify research on the influence of various abiotic degradation factors and the interplay between abiotic and biodegradation of PBAT.
Declaration of competing interest
The authors declare that they have no known competing financial interests or personal relationships that could have appeared to influence the work reported in this paper.
CRediT authorship contribution statement
Haibo Ye: Data curation, Investigation, Validation, Writing – original draft. Qianyu Li: Writing – review & editing. Juan Li: Writing – review & editing. Didi Li: Writing – review & editing. Zhimin Ao: Conceptualization, Funding acquisition, Resources, Supervision, Writing – review & editing.
Acknowledgments
This work was financially supported by the National Key R&D Program of China (No. 2022YFC3901800), the National Natural Science Foundation of China (No. 22176041) and Guangzhou Science and Technology Planning Project (No. 2023A04J0918).
-
-
[1]
J. Wang, Z. Tan, J. Peng, Q. Qiu, M. Li, Mar. Environ. Res. 113 (2016) 7–17. doi: 10.1016/j.marenvres.2015.10.014
-
[2]
J.C. Prata, A.L. Patricio Silva, J.P. da Costa, et al., Int. J. Environ. Res. Public Health 16 (2019) 2411. doi: 10.3390/ijerph16132411
-
[3]
H. Tian, Z. Tang, X. Zhuang, X. Chen, X. Jing, Prog. Polym. Sci. 37 (2012) 237–280. doi: 10.1016/j.progpolymsci.2011.06.004
-
[4]
T.P. Haider, C. Voelker, J. Kramm, K. Landfester, F.R. Wurm, Angew. Chem. Int. Ed. 58 (2019) 50–62. doi: 10.1002/anie.201805766
-
[5]
S. Aubin, J. Beaugrand, M. Berteloot, et al., Environ. Sci. Policy 134 (2022) 119–126. doi: 10.1016/j.envsci.2022.04.011
-
[6]
F.V. Ferreira, L.S. Cividanes, R.F. Gouveia, L.M.F. Lona, Polym. Eng. Sci. 59 (2019) E7–E15.
-
[7]
L. Jiang, M.P. Wolcott, J.W. Zhang, Biomacromolecules 7 (2006) 199–207. doi: 10.1021/bm050581q
-
[8]
European Bioplastics, Bioplastics facts and figures. European-bioplastics.org/ news/publications/, 2023 (accessed 3 January 2023)
-
[9]
M. Chen, C. Cai, J. Bao, et al., Polym. Degrad. Stabil. 203 (2022) 110080. doi: 10.1016/j.polymdegradstab.2022.110080
-
[10]
M. Zaverl, O. Valerio, M. Misra, A. Mohanty, J. Appl. Polym. Sci. 132 (2015) 1–11.
-
[11]
H. Hu, Y. Tian, J. Wang, R. Zhang, J. Zhu, Polym. Degrad. Stabil. 195 (2022) 109795. doi: 10.1016/j.polymdegradstab.2021.109795
-
[12]
S. Mohanty, S.K. Nayak, Polym-Plast. Technol. 50 (2011) 754. doi: 10.1080/03602559.2011.573395
-
[13]
L. Lai, S. Wang, J. Li, et al., Carbohyd. Polym. 247 (2020) 116687. doi: 10.1016/j.carbpol.2020.116687
-
[14]
Y. Fourati, Q. Tarres, M. Delgado-Aguilar, P. Mutje, S. Boufi, Int. J. Biol. Macromol. 183 (2021) 267–275. doi: 10.1016/j.ijbiomac.2021.04.102
-
[15]
J. Chen, R.R. Hu, F.L. Jin, S.J. Park, J. Appl. Polym. Sci. 138 (2021) 50250. doi: 10.1002/app.50250
-
[16]
B. Gewert, M.M. Plassmann, M. MacLeod, Environ. Sci-Proc. Imp. 17 (2015) 1513–1521.
-
[17]
Z. Lin, T. Jin, T. Zou, et al., Environ. Pollut. 304 (2022) 119159. doi: 10.1016/j.envpol.2022.119159
-
[18]
K. Zhang, A.H. Hamidian, A. Tubic, et al., Environ. Pollut. 274 (2021) 116554. doi: 10.1016/j.envpol.2021.116554
-
[19]
R.V. Moharir, S. Kumar, J. Clean. Prod. 208 (2019) 65–76. doi: 10.1016/j.jclepro.2018.10.059
-
[20]
F. Zhang, Y. Zhao, D. Wang, et al., J. Clean. Prod. 282 (2021) 124523. doi: 10.1016/j.jclepro.2020.124523
-
[21]
M. Musiol, W. Sikorska, G. Adamus, et al., Waste Manage 52 (2016) 69–76. doi: 10.1016/j.wasman.2016.04.016
-
[22]
T. Kijchavengkul, R. Auras, M. Rubino, M. Ngouajio, R.T. Fernandez, Chemosphere 71 (2008) 942–953. doi: 10.1016/j.chemosphere.2007.10.074
-
[23]
B. Laycock, M. Nikolic, J.M. Colwell, et al., Prog. Polym. Sci. 71 (2017) 144–189. doi: 10.1016/j.progpolymsci.2017.02.004
-
[24]
M.A. Maurer-Jones, E.M. Monzo, ACS Appl. Polym. 3 (2021) 1003–1011. doi: 10.1021/acsapm.0c01283
-
[25]
A. Kanwal, M. Zhang, F. Sharaf, C. Li, Polym. Bull. 79 (2022) 10053–10076. doi: 10.1007/s00289-021-03992-4
-
[26]
R. Herrera, L. Franco, A. Rodríguez-Galán, J. Puiggalí, J. Polym. Sci. A. Polym. Chem. 40 (2002) 4141–4157. doi: 10.1002/pola.10501
-
[27]
H. Wang, J. Wu, S. Chen, W. Xia, Chin. J. Biotechnol. 39 (2023) 1987–1997.
-
[28]
T. Kijchavengkul, R. Auras, M. Rubino, et al., Polym. Degrad. Stabil. 95 (2010) 2641–2647. doi: 10.1016/j.polymdegradstab.2010.07.018
-
[29]
Y.M. Bi, X.Y. Gong, W.Z. Wang, et al., Chin. Chem. Lett. 21 (2010) 237–241. doi: 10.1016/j.cclet.2009.10.003
-
[30]
Y. Ren, J. Hu, M. Yang, Y. Weng, J. Polym. Environ. 27 (2019) 2784–2792. doi: 10.1007/s10924-019-01563-3
-
[31]
Y.X. Weng, Y.J. Jin, Q.Y. Meng, et al., Polym. Test. 32 (2013) 918–926. doi: 10.1016/j.polymertesting.2013.05.001
-
[32]
L.Z. Zuo, H.X. Li, L. Lin, et al., Chemosphere 215 (2019) 25–32. doi: 10.1016/j.chemosphere.2018.09.173
-
[33]
Y. Fu, G. Wu, X. Bian, J. Zeng, Y. Weng, Molecules 25 (2020) 3946. doi: 10.3390/molecules25173946
-
[34]
X.F. Wei, M. Bohlen, C. Lindblad, M. Hedenqvist, A. Hakonen, Water Res. 198 (2021) 117123. doi: 10.1016/j.watres.2021.117123
-
[35]
Z.Y. Wei, L. Liu, J. Gao, P. Wang, M. Qi, Chin. Chem. Lett. 19 (2008) 363– 366. doi: 10.1016/j.cclet.2008.01.004
-
[36]
Q. Zhou, J. Zhang, M. Zhang, et al., Water Res. 222 (2022) 118899. doi: 10.1016/j.watres.2022.118899
-
[37]
A. Larranaga, E. Lizundia, Eur. Polym. J. 121 (2019) 109296. doi: 10.1016/j.eurpolymj.2019.109296
-
[38]
S. Jaiswal, B. Sharma, P. Shukla, Environ. Technol. Innovation 17 (2020) 100567. doi: 10.1016/j.eti.2019.100567
-
[39]
R.M.S. Cruz, V. Krauter, S. Krauter, et al., Foods 11 (2022) 3087. doi: 10.3390/foods11193087
-
[40]
A. Chamas, H. Moon, J. Zheng, et al., ACS Sustainable Chem. Eng. 8 (2020) 3494–3511. doi: 10.1021/acssuschemeng.9b06635
-
[41]
N. Lucas, C. Bienaime, C. Belloy, et al., Chemosphere 73 (2008) 429–442. doi: 10.1016/j.chemosphere.2008.06.064
-
[42]
R. Al-Itry, K. Lamnawar, A. Maazouz, Polym. Degrad. Stabil. 97 (2012) 1898–1914. doi: 10.1016/j.polymdegradstab.2012.06.028
-
[43]
R. Muthuraj, M. Misra, A.K. Mohanty, J. Appl. Polym. Sci. 132 (2015) 27.
-
[44]
A. Gopferich, Biomaterials 17 (1996) 103–114. doi: 10.1016/0142-9612(96)85755-3
-
[45]
M.F. Shockley, A.H. Muliana, Polym. Degrad. Stabil. 180 (2020) 109298. doi: 10.1016/j.polymdegradstab.2020.109298
-
[46]
Q. Deshoulles, M.Le Gall, S. Benali, et al., Polym. Degrad. Stabil. 205 (2022) 110122. doi: 10.1016/j.polymdegradstab.2022.110122
-
[47]
H. Jia, M. Zhang, Y. Weng, et al., J. Environ. Sci. 103 (2021) 50–58. doi: 10.1016/j.jes.2020.10.001
-
[48]
F. Muroi, Y. Tachibana, P. Soulenthone, et al., Polym. Degrad. Stabil. 137 (2017) 11–22. doi: 10.1016/j.polymdegradstab.2017.01.006
-
[49]
T. Kijchavengkul, R. Auras, M. Rubino, et al., Polym. Degrad. Stabil. 96 (2011) 1919–1926. doi: 10.1016/j.polymdegradstab.2011.07.001
-
[50]
D. Tang, C. Zhang, Y. Weng, Polym. Test. 99 (2021) 107204. doi: 10.1016/j.polymertesting.2021.107204
-
[51]
S. Majhi, Photoch. Photobio. Sci. 20 (2021) 1357–1378. doi: 10.1007/s43630-021-00100-3
-
[52]
T. Kijchavengkul, R. Auras, M. Rubino, M. Ngouajio, R.T. Fernandez, Chemosphere 71 (2008) 1607–1616. doi: 10.1016/j.chemosphere.2008.01.037
-
[53]
J. Xie, Y. Yan, S. Fan, et al., Environ. Sci. Technol. 56 (2022) 9041–9051. doi: 10.1021/acs.est.2c01687
-
[54]
T. Kijchavengkul, R. Auras, M. Rubino, et al., Polym. Degrad. Stabil. 95 (2010) 99–107. doi: 10.1016/j.polymdegradstab.2009.11.048
-
[55]
P. Stloukal, V. Verney, S. Commereuc, et al., Chemosphere 88 (2012) 1214–1219. doi: 10.1016/j.chemosphere.2012.03.072
-
[56]
G.X. De Ho, M.T. Zumstein, G.J. Getzinger, et al., Environ. Sci. Technol. 53 (2019) 2472–2481. doi: 10.1021/acs.est.8b06458
-
[57]
D. Briassoulis, J. Polym. J. Polym. Environ. 12 (2004) 65–81. doi: 10.1023/B:JOOE.0000010052.86786.ef
-
[58]
Y. Yang, C. Zhang, Y. Weng, Polym. Test. 102 (2021) 107334. doi: 10.1016/j.polymertesting.2021.107334
-
[59]
R.M. Qiao, C.P. Zhao, J.L. Liu, M.L. Zhang, W.Q. He, Polymers-Basel 14 (2022) 1434. doi: 10.3390/polym14071434
-
[60]
H. Jeswani, C. Krueger, M. Russ, et al., Sci. Total J. Polym. Environ. 769 (2021) 144483. doi: 10.1016/j.scitotenv.2020.144483
-
[61]
T. Maqsood, J. Dai, Y. Zhang, M. Guang, B. Li, J. Anal. Appl. Pyrol. 159 (2021) 105295. doi: 10.1016/j.jaap.2021.105295
-
[62]
S. Kim, W. Yang, H.S. Lee, Y.F. Tsang, J. Lee, J. Clean. Prod. 372 (2022) 133763. doi: 10.1016/j.jclepro.2022.133763
-
[63]
A.Felipe Jaramillo, S. Riquelme, L.Felipe Montoya, et al., Polym. Compos. 40 (2019) 1870–1882. doi: 10.1002/pc.24949
-
[64]
D.V.A. Ceretti, M. Edeleva, L. Cardon, D.R. D’Hooge, Molecules 28 (2023) 2344. doi: 10.3390/molecules28052344
-
[65]
F. Signori, M.B. Coltelli, S. Bronco, Polym. Degrad. Stabil. 94 (2009) 74–82. doi: 10.1016/j.polymdegradstab.2008.10.004
-
[66]
D.R. Tyler, J. Macromol. Sci-Pol. R. 44 (2004) 351–388. doi: 10.1081/MC-200033682
-
[67]
X. Li, Z. Cai, X. Wang, et al., Appl. Clay Sci. 196 (2020) 105762. doi: 10.1016/j.clay.2020.105762
-
[1]
-
Figure 4 Reaction mechanism between PBAT with (a) low and (b) high content of ADR (a chain extender). Reprinted with permission [50]. Copyright 2021, Elsevier.
Figure 5 Mechanisms involved in the photochemical transformation of PBAT. Reprinted with permission [24]. Copyright 2021, American Chemical Society.
Table 1. Proportions of different components of PBAT before and after degradation [33].
Table 2. Changes in molecular weight of the biodegradable films [22].
-

计量
- PDF下载量: 3
- 文章访问数: 700
- HTML全文浏览量: 22